Natural Selections Interviews Rockefeller’s Viviana Risca, Principal Investigator of the Laboratory of Genome Architecture and Dynamics
Alice Gadau
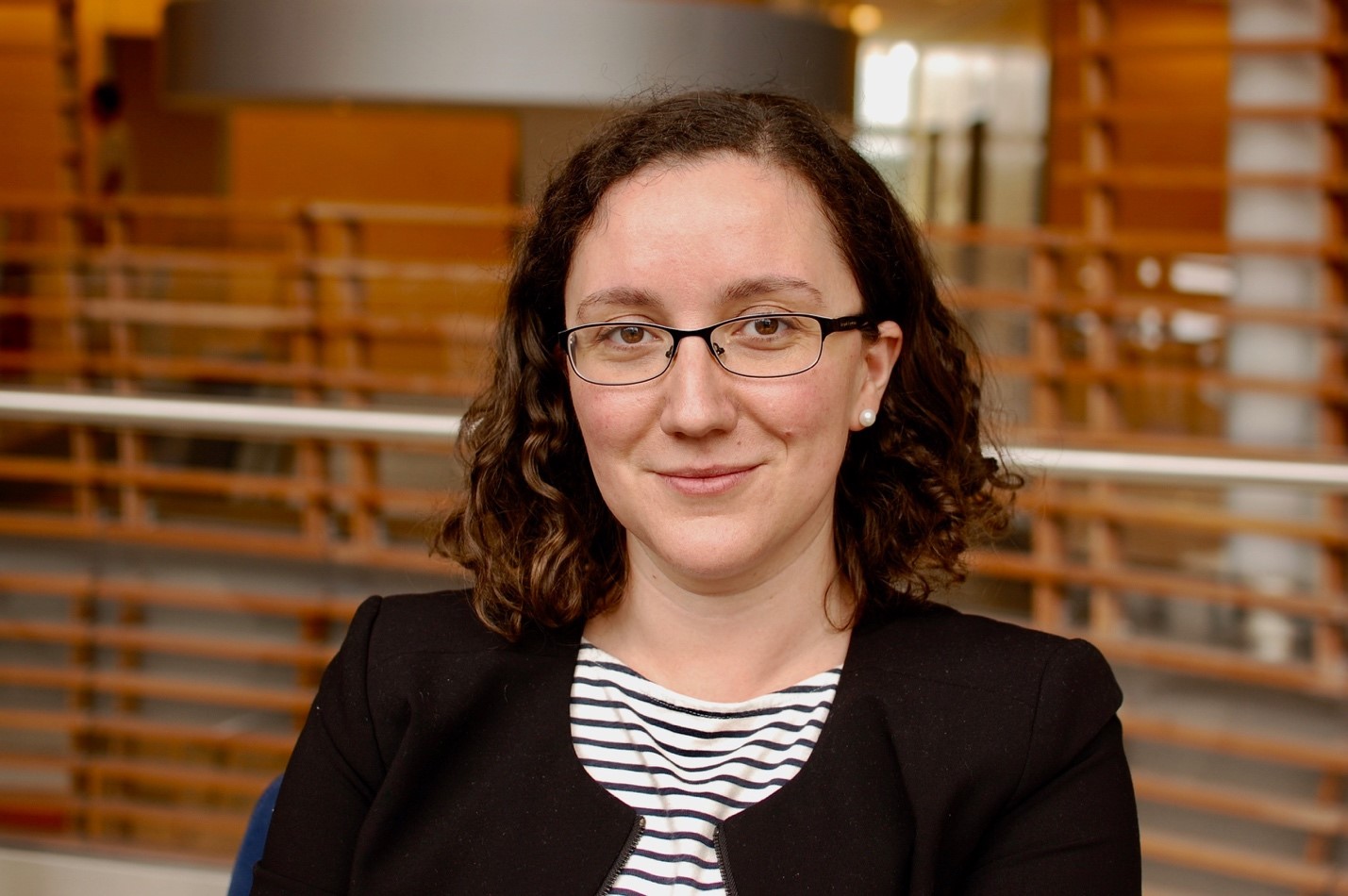
At the age of fourteen, Viviana Risca spent her summer break unlike most teenagers. Every morning, she took the train from Long Island to work in Dr. Daniel Eichinger’s parasitology laboratory at New York University. Despite the cabinet full of dead worms in formaldehyde she had to pass every morning, this first exposure to biological research was highly influential in propelling her into a lifetime of research. Since then she has been excited about using molecular techniques to understand the processes that occur in cells.
Viviana has now joined the Rockefeller community as one of the two new tenure-track assistant professors hired in 2018. After completing her Ph.D. in Biophysics at the University of California, Berkeley, she continued her scientific career as a postdoctoral scholar at Stanford University with Professor William J. Greenleaf. Viviana is interested in the biophysical rules that contribute to the dynamics and stability of the genome. In addition, she wants to understand how the three-dimensional genome architecture can regulate DNA-based processes.
Alice Gadau: What is the central question you are trying to address in your lab, and how is this an expansion from your past work?
Viviana Risca: The three-dimensional structure of the genome is controlled by its association with a variety of proteins, together making up a DNA-protein complex we call chromatin. Through the efforts of large-scale sequencing projects, we now know much of the linear sequence of bases that make up the human genome, but we are still far from fully understanding the mechanisms that control how and when the information encoded in DNA sequence is read out by transcription. A skin cell and a muscle cell contain the same genome, but they express different sets of genes that give rise to their unique identities.
My lab seeks to understand how three-dimensional chromatin structure controls the ways in which various proteins, including those involved in transcription, access their binding sites on DNA in order to perform their biological functions. One of the driving hypotheses of our work is that chromatin structure acts as an integrator of many molecular inputs to modulate access to DNA. I am particularly interested in how chromatin modifications, inter-molecular interactions, and polymer effects give rise to specialized parts of the cell’s nucleus that stably repress transcription and help maintain cell identity and normal cell function. Both chromatin structure and the processes that shape it are often perturbed in cancer, as well as during aging, and I hope that our work on these fundamental processes will provide useful leads toward better cancer therapies and diagnostics.
As a postdoc at Stanford, I worked on exploring how the landscape of enzyme-accessible chromatin can give us insights into the regulatory networks that control gene expression in several cell types, including a cancer model, using a popular method called ATAC-seq. I also did a lot of technology development, which resulted in RICC-seq, a method for mapping chromatin fiber structure at high resolution, and ChAR-seq, a method for mapping contacts between RNA and DNA genome-wide. In my own lab, we will continue to use and develop these technologies, applying them toward the goal of understanding the basic biophysical and molecular mechanisms responsible for stable transcriptional repression and overall control of access to genomic DNA.
AG: How does three-dimensional genome architecture contribute to DNA regulatory processes?
VR: Since the 1920s when Emil Heitz coined the terms euchromatin and heterochromatin, we have known that the cell nucleus is divided between regions of high and low chromatin density. Since then, we have learned that organization of chromatin is far from random—it is in fact organized at multiple length scales.
At short length scales, every 150-200 bp of eukaryotic genomes is wrapped around roughly 10 nm-wide histone complexes called nucleosomes. Regions of DNA that are depleted of nucleosomes tend to be hyper-accessible to exogenous enzymes we use to probe them, and we interpret these regions to be also accessible to endogenous proteins like transcription factors, chromatin remodelers and polymerases. Indeed, many of these proteins seem to localize to nucleosome-depleted DNA. We also know that some histone modifications, like lysine acetylation, is associated with low-density, euchromatic regions of the genome that tend to be more accessible to protein binding. The high-density, heterochromatic parts of the genome are less well understood, from a structural standpoint, and many labs, including my own, are currently trying to decipher the mechanisms that drive the exclusion of transcriptional machinery from these silent genomic regions. Candidate mechanisms that have been proposed include occlusion of binding sites by DNA buried in compacted chromatin, chemical exclusion by phase-separated liquid domains, and simple volume exclusion from high density regions.
We also know that there is functional communication between genomic regions at much longer length scales spanning tens of thousands and sometimes hundreds of thousands of kilobases. Enhancer elements in the genome, which are acetylated, hyperaccessible, and bound by transcription factors when active, somehow must communicate with gene promoter regions because they regulate these promoters. This is thought to happen via DNA looping interactions in three dimensions, but the exact nature and dynamics of these contacts are an active area of research and not yet understood. Lastly, we also know that the cell nucleus is divided up into regions with different functional profiles, through associations with the nuclear lamina or self-association of potentially phase-separated domains like the nucleolus. How this long-range organization relates to short-range chromatin folding is also an area that my lab plans to explore.
AG: What is RICC-seq and how can it accurately examine short range DNA contacts?
VR: RICC-seq stands for ionizing Radiation-Induced Correlated Cleavage. In the 1990s, it was shown that the pattern of DNA damage induced by X-rays or gamma-rays on a cell’s genome is shaped by the way the DNA is folded into chromatin. RICC-seq takes advantage of this convenient property of chromatin. We irradiate cells with X-rays or gamma rays, carefully extract the short pieces of resulting single-stranded DNA, and then sequence those pieces. Their ends tell us about the pattern of DNA damage and consequently, about the way the DNA was folded in the original cell. This method works well for mapping high-resolution DNA-DNA contacts on the order of less than 10 nm and less than a thousand base pairs, making it complementary to other three-dimensional structure mapping methods like Hi-C, which work better for contacts spanning thousands of kilobases.
AG: What makes RICC-seq novel from other sequencing techniques?
VR: RICC-seq has two unique advantages. First, it can be performed on living cells that are not fixed or permeabilized. Therefore, we can probe chromatin structure in its native state. Second, it uses hydroxyl radicals generated by radiation as its probe. The radiation can penetrate all areas of the nucleus, no matter how dense, and the radicals are smaller than water and can easily diffuse. Therefore, we can get data from not only the low-density euchromatin in the nucleus, but also from highly compacted, dense heterochromatin.
AG: What other tools are you incorporating in your lab to understand genome architecture?
VR: RICC-seq is not a single-cell method and requires quite a bit of input material, so we will be combining it with lower-input methods like single cell or bulk ATAC-seq and RNA-seq, as well new cutting-edge technologies as they emerge. We will also be complementing this work with protein binding measurements like ChIP-seq or CUT&RUN, and microscopy that can tell us about the long-range organization of the nucleus. Lastly, we will also be collaborating with chromatin engineers to develop perturbations that will help us dissect causal and functional relationships between molecular factors and chromatin structure.
AG: Are there other hobbies you have that incorporate science?
VR: I’ve long loved incorporating skills that I pick up in the lab into my other hobbies. When I learned microscopy and optics, I also took up photography in my spare time, because the basic concepts are the same. I love art in general and see a lot of parallels between the lives of scientists and artists. We are both seeking fundamental truths, and both need to continually find ways to nurture our creativity. I also love to cook. I often use it to relax because it uses many of the same skills as biochemistry or molecular biology, but you can usually determine failure or success more rapidly than at the bench, and it is also easier to share your successes with your friends.
Lastly, I love science outreach and communications, but I would not call those hobbies. I think all of us as scientists have a responsibility to share our work with the public that, after all, supports our work through taxes and donations, to advocate for the societal benefits of basic research, and to equitably educate the next generation.